From surviving desiccation to causing disease: Pathogenesis of Bacillus anthracis
Abstract:
Bacillus anthracis has wide ranging relevance historically and contemporarily, being as notable for its role in scientific discovery as it is for its role as a biological weapon in modern times. The aim of this review was to provide a comprehensive description of the processes involved in its pathogenesis. Using PubMed and Science Direct databases, sixteen primary research papers published after 2000 were identified for use here. Each article investigated a different aspect in the development of infection from spore germination to host death. Unlike other spore-forming bacteria, colonization and infection by B. anthracis cannot occur from vegetative cells and begins with germination of spores. Virulence factors are contained on plasmids pXO1 and pXO2, encoding for capsule and toxin production which work in tandem to evade the immune system while causing cellular destruction in target tissues including the heart and liver. This unique mechanism of causing disease results in delayed pathophysiological changes which complicate prompt diagnosis and may delay treatment, potentially increasing associated morbidity and mortality. The continued threat posed by this bacterial species, and the challenges it poses to healthcare, underscore the importance of understanding its pathogenesis fully.
Introduction:
The causative agent of anthrax, Bacillus anthracis, is a large, Gram-positive, rod-shaped species of bacteria that grows in chains and forms endospores (1). It is found in the environment in soil as spores where it can resist desiccation for decades (2, 3). It is a non-motile, obligate or facultative aerobe that grows in irregular, non-hemolytic, cream or white colonies that may have a mucoid appearance due to the presence of a capsule in some strains (2). It is most closely related to Bacillus cereus and Bacillus thuringiensis, which are also naturally found in the soil (3). All three species are infectious, with B. cereus and B. anthracis being clinically important to humans while B. thuringiensisinfects insects making it useful as an insecticide (4).
Anthrax is naturally a zoonotic infection especially in grazing animals, with transmission occurring from animals to humans (2). Infection can occur through direct contact, ingestion or inhalation of spores (2). There are three main presentations of anthrax infection which are dependent on the method of transmission (1). Spores transmitted through direct contact with broken skin will cause cutaneous anthrax in both humans and animals (2). This is the form of anthrax that the disease and bacteria were originally named for, classically presenting with black lesions on the skin (1). The ingestion of spores results in gastrointestinal anthrax, while the breathing in spores results in inhalation anthrax (1, 2). Both gastrointestinal and inhalation anthrax are rare as spores are less likely to be airborne naturally, but human activities have made these types of anthrax more common (5).
B. anthracis was first described clinically in 1752 and has been an integral part of several scientific advancements (6). Robert Koch used B. anthracis in his experiments to conclusively prove the germ theory of disease through his four postulates in 1876 (6). Building upon this work, Louis Pasteur created the first live, attenuated vaccine using B. anthracis in 1881(2, 6). This vaccine was used to prevent anthrax in livestock (2). Outside of these historical advancements in science, B. anthracis is most widely known as a weapon of bioterrorism following its use in the Amerithrax case of 2001 (7).
Pathogenesis
There are three main types of disease caused by infection from B. anthracis: cutaneous, gastrointestinal and inhalation (2). The type of disease that develops is dependent on the method of transmission and where the infection occurs (2, 8). Infection through abrasions on skin will result in cutaneous anthrax (2, 8). Ingestion through swallowing of spores results in gastrointestinal anthrax (2, 8). Finally, breathing in airborne spores results in inhalation anthrax which is the most lethal form (2, 8).
Cutaneous anthrax is the most common and least severe form of the disease (8). It produces black lesions at the affected sites, which are most commonly the head and extremities (2). Along with theses lesions, there will be localized inflammation and possible swelling of lymph nodes proximal to the site (2). Symptoms begin one to seven days after exposure, which is most often from handling of contaminated animals or animal products (2). It accounts for at least 90% of anthrax cases and is easily treated with antibiotics (8). The mortality rate is approximately 20% when left untreated (8).
There was an outbreak of anthrax in 2009 related to the use of intravenous drugs (8). Injection anthrax was identified in heroin users of Scotland, likely due to the use of contaminated heroin (8). Similar to cutaneous anthrax, this type of anthrax mainly affects the skin although there may be muscle involvement due to the nature of injection (1). The infection may also spread more quickly which could hamper timely identification and treatment, especially in a population that is often underserved by the medical community and prone to skin infections at injection sites (1). For this reason, the mortality rate of injection anthrax may be higher than that of cutaneous anthrax despite the similarities in symptoms and pathogenesis (1).
Gastrointestinal anthrax can also be transmitted through contaminated animals or animal products (1, 2). Consumption of contaminated meat is most often the mode of transmission for this presentation (2). It is characterized by loss of appetite, fever, headache, flushed face, sore throat, painful swallowing, abdominal pains, gastrointestinal inflammation, nausea, diarrhea and vomiting (1). Both vomit and stool may contain blood (1). Patients with gastrointestinal anthrax may develop lesions in their mouth, throat, stomach and intestines (2). Once established, the infection and toxins may spread into the blood stream (2). Infections develop one to seven days after exposure and there is an approximately 60% survival rate when properly treated (1).
Inhalation anthrax takes longer to develop, with symptoms beginning approximately one week to two months after exposure (2). Inhalation of airborne spores allow the bacteria to enter the respiratory tract where they begin by infecting the lymph nodes before spreading throughout the body (1). Symptoms include fever, body aches, headache, chills, excessive sweating, extreme fatigue, cough, chest discomfort, dizziness and shortness of breath (1). Almost all cases of inhalation anthrax are fatal if left untreated, and there is a mortality rate of 45% even with aggressive treatment (1).
Regardless of the route of transmission or type of disease, infection begins when the spores enter the body and germinate (9). It is important to note that infections from B. anthracis begin from spores and not vegetative cells (9). The presence of the spore is essential to the survival of this bacteria by preventing desiccation, but also plays an integral role in its pathogenicity (9). Using a mouse model, Bischof, et al. demonstrated that cutaneous anthrax occurred only when spores were inoculated onto abraded skin (10). Inoculation of abraded skin with vegetative cells did not result in infection (10). The inability for vegetative cells to cause infection prevents person-to-person transmission (1).
An asymmetrical septum divides the spore into a smaller forespore and a larger mother cell during sporulation (11). The forespore then develops a two-layered, compact, proteinaceous protective coat (11). Giorno, et al, determined this coat was not a virulence factor for B. anthracis despite the critical role of spores in infection (11). Ungerminated spores have been found to contain protective antigen (PA), an essential component for anthrax toxicity (9). This may play a role in the uptake of spores by macrophages and subsequent intracellular germination (9).
Germination is initiated once the spore is in a nutrient-rich environment, prompted by the binding of germinants to receptors on the spore (2). Spores are ingested by macrophages or dendritic cells at the site of infection and are transported to the efferent lymph nodes (2). In the case of inhalation anthrax, the site of infection becomes the mediastinal lymph nodes, not the lungs (2). Inside the macrophage, germinated spores begin producing lethal factor (LF) within three hours following infection (9).
There are two exotoxins involved in B. anthracis pathogenesis. Anthrax lethal factor (LF) and anthrax edema factor (EF) play a role in establishing infection and causing disease. In addition, both toxins require protective antigen (PA) to gain entry to target cells (9, 12). The combination of PA with EF or LF creates edema toxin (ET) and lethal toxin (LT) respectively (12). These toxins work in tandem as the bacteria begins to establish within the host, impairing the host immune cells and limiting the immune response (13).
Capsule formation and toxin production are encoded on two plasmids, pXO1 and pXO2 (12). Encapsulation occurs following germination of spores and allows systemically circulating vegetative cells to resist phagocytosis (14). Activation of the cap and dep genes initiates the production, degradation, and release of the capsule polymers (14). Makino, et al. investigated the role of the capsule in pathogenesis, including the development of the low molecular weight capsule (L-capsule) from degradation of the high molecular weight capsule (H-capsule) (14). They found that the L-capsule protects against complement activation by vegetative cells while also inducing its release to evade phagocyte-mediated killing (14).
The genes for PA (pag), EF (cya), and LF (lef) are encoded for on plasmid pXO1 (12). The capsular proteins are encoded for on pXO2 (12). Both LF and EF work by disrupting cell signaling through binding with their respective targets (12).The target of metalloprotease LF is mitogen-activated protein kinase (MAPK) kinases (MAPKKs), while EF is a calmodulin-dependent adenylate cyclase that increases the concentration of intracellular cyclic adenosine monophosphate (cAMP) (12, 13).
PA binds to two cellular receptors: tumor endothelium marker-8 (TEM8), also known as anthrax toxin receptor 1 (ANTXR1), and capillary morphogenesis protein-2 (CMG2), also known as anthrax toxin receptor 2 (ANTXR2) (15). After PA binds to these receptors, it is cleaved, and the amino-terminal dissociates while the carboxyl-terminal heptamerizes (16). LF and EF bind individually to the bound PA and are translocated to the cytosol of the cell from the phagolysosome (9, 15). Rainey, et al. found release of PA from the receptor results in pore formation for toxin translocation (17). Once in the cytosol, cellular intoxication occurs and results in cell death (15).
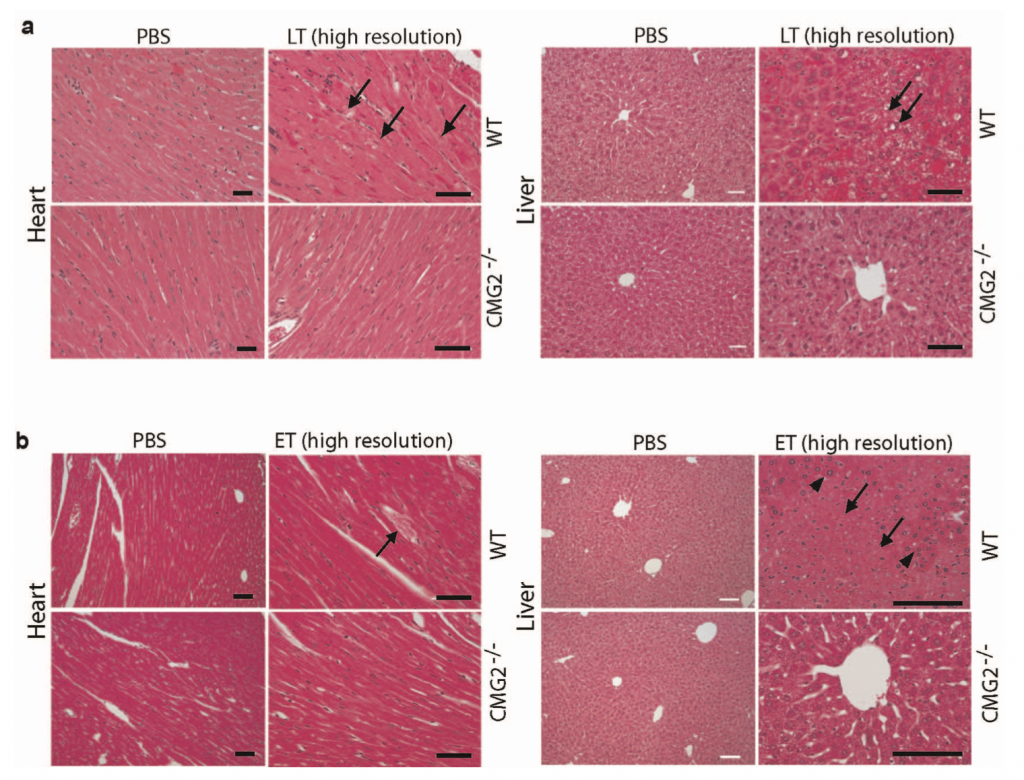
Figure 1: Heart and liver histology of ET and LT-treated wild-type and mutant mice models showing areas of degeneration (15). |
Vegetative cells continue to replicate, forming short chains, and produce toxins that begin to circulate systemically (9). Investigations by Liu, et al, showed that the target for LT is cells of cardiac and smooth muscle (15). Damage to hepatocytes from LT was demonstrated to be secondary to lethality induced in cardiomyocytes (15). However, ET was found to target hepatocytes and intestinal epithelium resulting in fluid accumulation (15). In combination, these toxins lead to system collapse through destruction of the cardiovascular system and liver (15). Figure 1 depicts the histological effects of LT and ET in mouse models (15). Additionally, LT efficiently kills murine macrophage lines and dendritic cells which disrupts the host ability to respond to infection (13).
The ability of B. anthracis to infect and kill immune cells responding to the infection contributes to the proliferation of cells and production of toxins (13). Toxin production within macrophages inhibits the host immune response in two phases (13). Release of LT inactivates the MAPK pathways, inducing cleavage of MEK1 in alveolar macrophages (AM) which impairs cytokine secretion (13, 18). This is part of the inactivation phase which modulates the immune responses and facilitates bacterial survival (13, 18). The second phase involves the killing of dendritic cells, the mechanism of which is dependent on the genetic lineage of the affected cell, which enables cell proliferation (13).
The pathophysiological changes resulting from B. anthracis infection are nonspecific and have a delayed presentation (19). A significant increase in white blood cells is observed at the onset of septicemia, with a median white blood cell count of 9.8×103/mm3 (16, 19). Levels of calcium, sodium, and cholinesterase are lowered, while potassium, chloride, phosphate and serum transaminase are elevated (16, 19). Marked hypoglycemia is observed following the onset of septicemia and may contribute to death of the host (19). Additionally, increased blood pH and reduced blood oxygen levels have been observed (19).
Control Methods:
Treatment of anthrax involves the use of antibiotics as well as supportive therapy, depending on the type of disease (20). B. anthracis is susceptible to penicillins, fluoroquinones and tetracyclines, though some strains have exhibited penicillin resistance as seen in Figure 2 (20). Depending on the severity of disease, antibiotics may need to be administered intravenously and hospitalization may be required (2, 21). Additionally, antitoxins that counteract the toxins produced by B. anthracis are available for administration to limit the effects of infection (21). In the event of exposure, a two-month prophylactic course of antibiotics is used to prevent the development of infection (2).
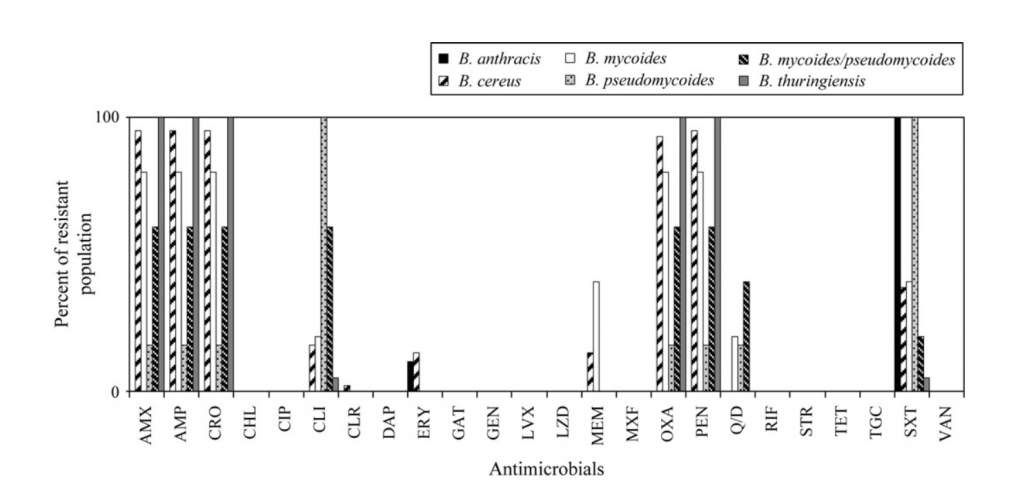
Figure 2: Percentage of Bacillus species showing resistance to antimicrobials based on MIC. AMX, amoxicillin; AMP, ampicillin; CRO, ceftriaxone; CHL, chloramphenicol; CIP, ciprofloxacin; CLI, clindamycin; CLR, clarithromycin; DAP, daptomycin; ERY, erythromycin; GAT, gatifloxacin; GEN, gentamicin; LVX, levofloxacin; LZD, linezolid; MEM, meropenem; MXF, moxifloxacin; OXA, oxacillin; PEN, penicillin; Q/D, quinupristin/dalfopristin; RIF, rifampicin; STR, streptomycin; TET, tetracycline; TGC, tigecycline; SXT, trimethoprim/sulfamethoxazole; VAN, vancomycin (20). |
Anthrax prevention strategies include decontamination and vaccination (2, 22). Limiting exposure through decontamination can be utilized against both naturally-occurring and laboratory-created strains (22). Decontamination can occur through chemical or physical methods, including disinfectants and UV light (22, 23). Wood, et al. found that exposure to UVC from an LED source in low humidity environments was the most effective method of inactivation by light (22). However, UV light was not found to achieve the same levels of log reduction achieved by chemical methods (22).
Vaccination is available for both animals and humans (2). The human anthrax vaccine is only administered to those at high risk of exposure such as laboratory workers who handle B. anthracis or certain members of the military in the United States (24). It can be given before or after exposure to prevent the development of disease and is the most effective method for preventing large outbreaks (2, 24). The use of personal protective equipment when handling potentially contaminated items can further reduce exposure (25).
Future Outlook:
Historically, anthrax has been a disease affecting people in close contacts with animals or animal products, such as farm or textile industry workers (7). In 2001, the Amerithrax case brought B. anthracis and its potential as a biological weapon into the national spotlight (7). The ability of spores to be widely disseminated among public spaces poses a grave threat, while the accident at Sverdlovsk in 1979 demonstrates that other nations have worked to weaponize and refine B. anthracis for such a purpose (26, 27). In conjunction with the risk of bioterrorism, the response to an attack could significantly deplete the available supply of antibiotics used in treatment and prophylaxis (20). The development of antibiotic resistance in B. anthracis could pose an additional challenge in the future (20).
Along with manmade threats, the effects of global climate change could increase the risks of contracting anthrax from natural sources (28). Melting permafrost and retreating ice pack in the Arctic circle has led to uncovering of well-preserved carcasses (28). In Siberia, deer carcasses were found to be contaminated with spores of B. anthracis in several different layers of permafrost (28). While anthrax is currently endemic in Russia, confined to sporadic outbreaks in animals and humans, there is potential for increased exposure as temperatures in the Arctic Circle region continue to increase (5).
The outbreak of anthrax related to the use of intravenous drugs demonstrates the potential for human activity to create novel exposure events from naturally occurring sources (1). While the primary manifestation of this form resembled cutaneous anthrax, involvement of deeper tissues due to the nature of injections had the potential to complicate diagnosis (1). Several different infections are more common at injection sites, which could also delay proper identification of the causative agent (1). Increased awareness of alternative presentations among medical professionals, including emergency room and laboratory staff, can facilitate prompt diagnosis and treatment to improve patient prognosis (8).
Conclusions:
Bacillus anthracis is an important human and animal pathogen, both historically and presently (6). The route of transmission determines the form of anthrax that develops, either cutaneous, gastrointestinal, or inhalation, with cutaneous being the most common type of infection (1). Formation of the endospore is not only essential in preventing desiccation but has shown to be necessary for causing disease (9). Germination follows inoculation on abraded skin or uptake of spores by macrophages, utilizing the cells of the innate immune system to initiate infection (2, 10). Capsule formation and toxin production are encoded on the same plasmid, with both playing important roles in the pathogenesis (12). Binding of protective antigen (PA) to cellular receptors precedes entry of lethal toxin (LT) and edema toxin (ET) into the cytosol (17).
Disease progression involves cellular proliferation and release of toxins into the blood stream (2). LT and ET target cardiomyocytes and hepatocytes respectively, with significant cellular disruption contributing to host death (15). Treatment of all forms requires antibiotics, with ciprofloxacin being preferred, and administration of antitoxin being used in systemic cases (21). Prevention methods rely on decontamination, vaccination of at-risk individuals, protective precautions, and administration of prophylactic antibiotics following exposure (2, 24, 25). While naturally endemic to areas throughout the globe, the rising global temperature and the threat of use as a biological weapon pose a continued risk to humans (5, 26, 28).
References
1. Control CfD. Guide to Understanding Anthrax. 2016.
2. Spencer RC. Bacillus anthracis. J Clin Pathol. 2003;56(3):182-7. Epub 2003/03/01. doi: 10.1136/jcp.56.3.182. PubMed PMID: 12610093; PMCID: PMC1769905.
3. Koehler TM. Bacillus anthracis physiology and genetics. Mol Aspects Med. 2009;30(6):386-96. Epub 2009/08/06. doi: 10.1016/j.mam.2009.07.004. PubMed PMID: 19654018; PMCID: PMC2784286.
4. Ehling-Schulz M, Lereclus D, Koehler TM. The Bacillus cereus Group: Bacillus Species with Pathogenic Potential. Microbiol Spectr. 2019;7(3):7.3.6. Epub 2019/05/22. doi: 10.1128/microbiolspec.GPP3-0032-2018. PubMed PMID: 31111815; PMCID: PMC6530592.
5. Carlson CJ, Kracalik IT, Ross N, Alexander KA, Hugh-Jones ME, Fegan M, Elkin BT, Epp T, Shury TK, Zhang W, Bagirova M, Getz WM, Blackburn JK. The global distribution of Bacillus anthracis and associated anthrax risk to humans, livestock and wildlife. Nat Microbiol. 2019;4(8):1337-43. Epub 2019/05/16. doi: 10.1038/s41564-019-0435-4. PubMed PMID: 31086311.
6. Control CfD. History of Anthrax 2020. Available from: https://www.cdc.gov/anthrax/basics/anthrax-history.html.
7. Jernigan JA, Stephens DS, Ashford DA, Omenaca C, Topiel MS, Galbraith M, Tapper M, Fisk TL, Zaki S, Popovic T, Meyer RF, Quinn CP, Harper SA, Fridkin SK, Sejvar JJ, Shepard CW, McConnell M, Guarner J, Shieh WJ, Malecki JM, Gerberding JL, Hughes JM, Perkins BA. Bioterrorism-related inhalational anthrax: the first 10 cases reported in the United States. Emerg Infect Dis. 2001;7(6):933-44. Epub 2001/12/19. doi: 10.3201/eid0706.010604. PubMed PMID: 11747719; PMCID: PMC2631903.
8. Control CfD. Types of Anthrax 2020 [02/26/2022]. Available from: https://www.cdc.gov/anthrax/basics/types/index.html.
9. Cote CK, Welkos SL. Anthrax Toxins in Context of Bacillus anthracis Spores and Spore Germination. Toxins (Basel). 2015;7(8):3167-78. Epub 2015/08/20. doi: 10.3390/toxins7083167. PubMed PMID: 26287244; PMCID: PMC4549744.
10. Bischof TS, Hahn BL, Sohnle PG. Characteristics of Spore Germination in a Mouse Model of Cutaneous Anthrax. The Journal of Infectious Diseases. 2007;195(6):888-94. doi: 10.1086/511824.
11. Giorno R, Bozue J, Cote C, Wenzel T, Moody KS, Mallozzi M, Ryan M, Wang R, Zielke R, Maddock JR, Friedlander A, Welkos S, Driks A. Morphogenesis of the Bacillus anthracis spore. J Bacteriol. 2007;189(3):691-705. Epub 2006/11/23. doi: 10.1128/JB.00921-06. PubMed PMID: 17114257; PMCID: PMC1797280.
12. Levy H, Weiss S, Altboum Z, Schlomovitz J, Glinert I, Sittner A, Shafferman A, Kobiler D. Differential contribution of Bacillus anthracis toxins to pathogenicity in two animal models. Infect Immun. 2012;80(8):2623-31. Epub 2012/05/16. doi: 10.1128/IAI.00244-12. PubMed PMID: 22585968; PMCID: PMC3434592.
13. Alileche A, Serfass ER, Muehlbauer SM, Porcelli SA, Brojatsch J. Anthrax Lethal Toxin-Mediated Killing of Human and Murine Dendritic Cells Impairs the Adaptive Immune Response: e19. PLoS Pathogens. 2005;1(2). doi: 10.1371/journal.ppat.0010019. PubMed PMID: 1314350558.
14. Makino S, Watarai M, Cheun HI, Shirahata T, Uchida I. Effect of the lower molecular capsule released from the cell surface of Bacillus anthracis on the pathogenesis of anthrax. J Infect Dis. 2002;186(2):227-33. Epub 2002/07/23. doi: 10.1086/341299. PubMed PMID: 12134259.
15. Liu S, Zhang Y, Moayeri M, Liu J, Crown D, Fattah RJ, Wein AN, Yu ZX, Finkel T, Leppla SH. Key tissue targets responsible for anthrax-toxin-induced lethality. Nature. 2013;501(7465):63-8. Epub 2013/09/03. doi: 10.1038/nature12510. PubMed PMID: 23995686; PMCID: PMC4080305.
16. Bradley KA, Mogridge J, Mourez M, Collier RJ, Young JAT. Identification of the cellular receptor for anthrax toxin. Nature. 2001;414(6860):225. doi: 10.1038/n35101999. PubMed PMID: 5591988.
17. Rainey GJA, Wigelsworth DJ, Ryan PL, Scobie HM, Collier RJ, John ATY. Receptor-Specific Requirements for Anthrax Toxin Delivery into Cells. Proceedings of the National Academy of Sciences of the United States of America. 2005;102(37):13278-83; PMCID: PMC1201603.
18. Ribot WJ, Panchal RG, Brittingham KC, Ruthel G, Kenny TA, Lane D, Curry B, Hoover TA, Friedlander AM, Bavari S. Anthrax lethal toxin impairs innate immune functions of alveolar macrophages and facilitates Bacillus anthracis survival. Infect Immun. 2006;74(9):5029-34. Epub 2006/08/24. doi: 10.1128/IAI.00275-06. PubMed PMID: 16926394; PMCID: PMC1594833.
19. Klein F, Walker JS, Fitzpatrick DF, Lincoln RE, Mahlandt BG, Jones WI, Jr., Dobbs JP, Hendrix KJ. Pathophysiology of anthrax. J Infect Dis. 1966;116(2):123-38. Epub 1966/04/01. doi: 10.1093/infdis/116.2.123. PubMed PMID: 4956203.
20. Luna VA, King DS, Gulledge J, Cannons AC, Amuso PT, Cattani J. Susceptibility of Bacillus anthracis, Bacillus cereus, Bacillus mycoides, Bacillus pseudomycoides and Bacillus thuringiensis to 24 antimicrobials using Sensititre® automated microbroth dilution and Etest® agar gradient diffusion methods. Journal of Antimicrobial Chemotherapy. 2007;60(3):555-67. doi: 10.1093/jac/dkm213.
21. Control CfD. Treatment of Anthrax Infection 2020 [updated 11/20/2020]. Available from: https://www.cdc.gov/anthrax/treatment/index.html.
22. Wood JP, Archer J, Calfee MW, Serre S, Mickelsen L, Mikelonis A, Oudejans L, Hu M, Hurst S, Rastogi VK. Inactivation of Bacillus anthracis and Bacillus atrophaeus spores on different surfaces with ultraviolet light produced with a low-pressure mercury vapor lamp or light emitting diodes. J Appl Microbiol. 2020;n/a(n/a). Epub 2020/07/22. doi: 10.1111/jam.14791. PubMed PMID: 32692423.
23. Setlow B, Parish S, Zhang P, Li YQ, Neely WC, Setlow P. Mechanism of killing of spores of Bacillus anthracis in a high-temperature gas environment, and analysis of DNA damage generated by various decontamination treatments of spores of Bacillus anthracis, Bacillus subtilis and Bacillus thuringiensis. J Appl Microbiol. 2014;116(4):805-14. Epub 2013/12/19. doi: 10.1111/jam.12421. PubMed PMID: 24344920.
24. Control CfD. Vaccine to Prevent Anthrax 2020. Available from: https://www.cdc.gov/anthrax/prevention/vaccine/index.html.
25. Health TNIfOSa. Anthrax: Recommendations for Protecting Workers: Centers for Disease Control; 2014. Available from: https://www.cdc.gov/niosh/topics/anthrax/workers.html.
26. Committee on Review of the Scientific Approaches Used During the FsIotBAM, National Research C. Review of the Scientific Approaches Used during the FBI’s Investigation of the 2001 Anthrax Letters. 2011. doi: 10.17226/13098. PubMed PMID: 24983068.
27. Meselson M, Guillemin J, Hugh-Jones M, Langmuir A, et al. The Sverdlovsk anthrax outbreak of 1979. Science. 1994;266(5188):1202. PubMed PMID: 213540738; 02177820.
28. Timofeev V, Bahtejeva I, Mironova R, Titareva G, Lev I, Christiany D, Borzilov A, Bogun A, Vergnaud G. Insights from Bacillus anthracis strains isolated from permafrost in the tundra zone of Russia. PLoS One. 2019;14(5):e0209140. Epub 2019/05/23. doi: 10.1371/journal.pone.0209140. PubMed PMID: 31116737; PMCID: PMC6530834.